Once the sunlight is concentrated by concentrators, several methods can be used to convert the heat into electrical energy. The conventional approach is to use a heat engine.
Several power conversion methods have been developed for solar central receivers. The power conversion unit of large central receivers (20–200 MWe) is likely to be a steam Rankine turbine, while smaller central receivers can have intrinsically better optics and thus accommodate Brayton and combined cycles operating at higher temperatures. A 10-MWe system using molten salt as heat transfer fluid and storage medium, combined with a steam Rankine turbine at up to about 850K, has been demonstrated in the U.S. Department of Energy’s Solar II project. Other methods are (a) steam generation and superheating in the receiver, (b) heating atmospheric air to about 950K in the receiver and then using it to superheat steam, and (c) heating compressed air in the receiver to over 1100K and using it in a solar/fuel hybrid gas-turbine.
An important area that has not been sufficiently explored is the development of heat engines specifically designed for integration in a solar thermal system, as opposed to the customary approach of modifying existing engines. Such approach could take advantage of recent turbomachinery component developments (e.g., combustors, recuperators, alternators, bearings, ceramic rotors). The use of such engines instead of a modified existing fuel-driven engine should substantially simplify the power conversion unit, increase system efficiency, and lead to a significant cost reduction.
In general, new developments and innovations in the various methods described above can reduce the cost of solar thermal electricity production to about $2/W, or even less (e.g., in dish/engines). Such installed costs are low enough to provide cost-competitive electrical energy if fuel costs remain at their present value and carbon emission limitations are implemented.
Solar Thermoelectric Power Generators. Direct thermal-to-electric energy conversion engines based on thermoelectric devices and thermophotovoltaic (TPV) energy converters provide new opportunities for medium power ranges that may rival direct photovoltaic (PV) power conversion and involve no moving parts.
Thermoelectric energy conversion technology, based on the Peltier effect and the Seebeck effect, exploits the thermal energy of electrons (and holes) for the energy conversion between heat and electricity, including power generation, refrigeration, and heat pumping.
A thermoelectric power generator has a maximum efficiency given by
η=[(Th-Tc)/Th]*[√(1+ZTm-1)/(√(1+ZTM)+Tc/Th)]
where
Th,Tc = temperatures at the hot and cold sides
Tm = mean temperature
Z = measure of the electronic power produced by the thermal gradient, divided by the thermal conductivity.
A large thermal conductivity would degrade performance. The product of Z and the working temperature T forms a nondimensional figure of merit, ZT. With a value of ZT between 3 and 4, thermoelectric devices would have an efficiency approaching that of an ideal heat engine. Thus, the key for the thermoelectric technology is to find materials with ZT>3. Materials with reasonable ZT are often heavily doped semiconductors and some semimetals. The ZT value of a given material is temperature dependent; it usually peaks at certain temperature and drops off at higher temperatures.
The best commercial materials are alloys of Bi2Te3 with Bi2Se3 (n-type) and with Sb2Te3 (p-type). The alloys are used because the phonon thermal conductivity can be significantly reduced with only a small reduction in the electronic power factor. Bi2Te3-based alloys have a peak ZT around 1 near room temperature. Thus, these materials are not optimal for solar power production, where the operating temperatures are higher. Bi2Te3-based materials, used in some power generation applications, have a module efficiency that is limited to 5%. The U.S. National Aeronautics and Space Agency used SiGe alloys (and PbTe-based alloys) to make radioisotope powered thermoelectric power generators operating in the temperature range of 300–900°C (and 300–600°C for PbTe-based alloys), with a system conversion efficiency ~6–7%. These materials all have a maximum ZT less than but close to 1.
Commercial thermoelectric materials, with a maximum ZT~1, were mostly discovered in 1950s. Little progress was made in the subsequent years. In the 1990s, the possibility of improving the thermoelectric figure of merit based on electron band gap engineering and phonon engineering in nanostructures was investigated. These ideas have lead to a resurgence in thermoelectric research and significant progress in improving ZT, particularly based on nanostructured materials. Venkatasubramanian et al. reported that Bi2Te3/Sb2Te3-based p-type superlattices have a room-temperature ZT of 2.4. Harman et al. reported that PbTe/PbTeSe superlattices with nanodots formed by strain have a room-temperature ZT of 2.0. Hsu et al. reported bulk nanostructures of AgPb2SbTe2+m. with a ZT of 2.2 at 527°C. Meanwhile, several research projects aiming at improving device efficiency based on more mature materials are under way. The Jet Propulsion Laboratory reported a segmented thermoelectric unicouple with an efficiency of ~14% with the hot side at 975K and cold side at 300K. ATO Solar generators made of materials with ZT~4 operating between room temperature and 1000°C would reach an efficiency of 35%. Given the impressive development made in the field of thermoelectrics over the past decade, the development of such materials seems to be a realizable goal.
Solar Thermophotovoltaics. Solar TPVs are similar to solar cells in that they convert photon energy into electricity. The fundamental difference from other PVs is that the photon source comes from a terrestrial thermal radiation emitter rather than directly from the sun. The radiation emitter can be heated by thermal sources such as fuel combustion or by concentrated solar radiation. Solar TPVs have a theoretical system efficiency of >30% for a concentration ratio of >10,000. Compared with nonconcentrated solar PVs, the radiation emitted by the emitter has a higher power density. Thermal radiation from the heated radiation emitter has a longer wavelength and correspondingly, the PV cells used in a TPV system often have lower band gaps. Figure 15 shows a possible set-up of a solar TPV system. Concentrated solar energy raises the temperature of a solid thermal radiation emitter to a high temperature, typically to the range of 1,000– 2,000°C. The efficiency of TPV systems depends critically on spectral filtering to avoid absorption of photons with energies below the band gap of the PV cells by parts of the system other than the emitter. The filtering elements can be freestanding or integrated in the emitter or the cells. The efficiency of a TPV power generator system can be roughly split into several factors:
η=ηsourceηspectralηdiodeηmech
where
ηsource = efficiency of the conversion of the energy source (fossil, solar, nuclear) into thermal radiation from the emitter
ηspectral = combined efficiency of the emitter and filter that represents the fraction of photon energy above the band gap reaching the PV cell among all photon energy emitted
ηdiode = efficiency of the PV cell converting the photon energy above the band gap into electricity,
ηmech = efficiency of converting PV cell electrical power output to the system power output that includes the energy lost in the pumping systems for fuel injection and thermal management.
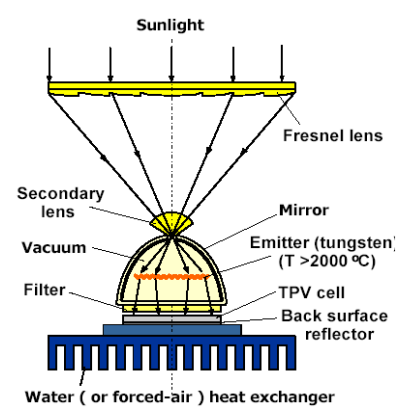
Thermophotovoltaic energy conversion emerged in 1950s through the work of Henry Kolm at the Massachusetts Institute of Technology Lincoln Laboratory and a series of lectures given by Pierre R. Aigrain of the École Normale Superieure. The focus of past work was on diode development and spectral control. Most diodes are built on antimony-based III-V materials with a band gap in the range of 0.4–0.7 eV. One mature example is GaSb-based TPV diodes, which were also used in high-efficiency tandem solar cells. Thin-filmbased diodes based on InGaAsSb are also extensively studied. The band gap of such thin-film materials can be tailored to match the heat-source temperature for optimum performance. Heterostructures can be used to further improve the cell performance. A 27% diode efficiency and 20% combined radiator-diode has been reported. With proper spectral control and further diode developments, solar TPV systems with efficiency in the range of 25–35% are possible.
Spectral control is of crucial importance and holds the key for TPV efficiency. The goal of spectral control is to allow only photons above the band gap to reach the diode, as photons below the band gap not only represent a loss of useful energy but also reduce diode efficiency because they cause a rise in the diode temperature when being absorbed. For emission control, rare earth and transition metal-doped ceramics, refractory intermetallic coatings, thin-film and multilayer filters, plasmonic filters, and photonic crystals have been explored. However, high-temperature operation of the emitters poses great challenges to the stability of the materials and structures. In comparison, filters, either stand-alone or built on the surface of the diode, suffer less from the stability issue.
In 2002, for a 1.5-kW GaSb-based system used as a home furnace, the total cost was estimated to be $4,200 with $2,700 for the furnace and $1,500 for the TPV generator at ~15% efficiency. This corresponds to $1/W. If we add in the cost of the concentrator at $1.6/W (assuming 15% efficiency, 850 W/m2 solar insolation, and $200/m2 concentrator cost), the cost is $2.6/W based on current technology (not counting other items that may be needed for the solar TPV system). If the efficiency is doubled to 30%, reducing the cost of energy in half, then the other major opportunity for cost reduction is the concentrator cost. This cost would need to be reduced significantly to bring the total cost to a target cost of $1/W as for solar PV.
Concentrated Photovoltaics. Concentrated photovoltaic systems do not involve a solar thermal process, but they share the concentrator issues of linear and central receivers. Thus, this fast developing field might well be considered under the “Crosscutting Areas” category. In this method, sunlight is concentrated by using mirrors or lenses, which are much cheaper than generator with solar panels, and the concentrated light is focused onto the PV cells. The required cell area is therefore reduced by the concentration factor, which in present systems can be as high as 500; future CPV systems may be able to sustain even higher concentration ratios. We refer the reader to the Basic Research Challenges for Solar Electricity and Solar Electricity Technology Assessment for details of PV development.